Diamonds are expensive: lessons learned about applying synthetic biology for 1000+ year carbon dioxide removal
Using biotechnology for long-term durable (1000+ years) carbon removal has been of interest for several years, although this field is still in its early stages. Proposed ideas have included the production of highly decay-resistant molecules (such as suberin, enhanced lignin, and sporopollenin), increased root biomass, bio-enhanced rock weathering and carbon mineralization, and hybrid approaches that use biology to enhance direct air capture (DAC).
At Living Carbon, we had experience engineering trees for enhanced photosynthesis and fungal decay resistance, but wanted to add a long-term durable carbon dioxide removal (CDR) approach to our portfolio. We applied to Stripe’s Spring 2022 carbon removal purchase cycle with an idea that we had been considering for a while: engineering increased production of sporopollenin. Otherwise known as the “diamond of the plant world,” sporopollenin is the main component of exine, the outer coating of most pollen grains and spores. It is the toughest biopolymer nature has ever produced and has been found in rocks over 500 million years old.

Our approach to choosing and developing the sporopollenin project
Our investigation of biological, durable CDR began by taking an inventory of the most recalcitrant forms of carbon storage existing in nature. We then focused on sporopollenin because of its high durability. We wanted to leverage the ability of biological systems to grow autotrophically, covering the “capture” part of carbon removal with a fast growth rate. Then, we would synthetically add the “storage” component to enable durable CDR at a theorized low cost. We assumed that by adding long-term storage to a rapid-growth organism, we could deliver 1000+ years of storage at less than $100/ton.
In retrospect, we believe this was not the correct starting point. Rather than starting with a list of potential solutions (in this case, durable substances that could be produced biologically), going forward, we would start by surveying the largest-scale and/or most efficient existing forms of CO₂ sequestration and questioning whether these processes could be biologically enhanced.

A year later, our science team had made impressive progress, but we concluded it would be near-impossible to produce sporopollenin at <$100/ton. We learned a lot about what it means to use synthetic biology for high-quality, low-cost carbon removal, and wanted to share these learnings with the broader community. There are certain commercial pitfalls that may be applicable to other proposed synbio-for-CDR solutions and are worth considering early in their evaluation. We believe solutions that clearly articulate a strategy to avoid these pitfalls are much more likely to succeed and to move the emerging field of synthetic biology for climate toward research that has the best chance of scaling.
During our initial evaluation of durable CDR ideas, we evaluated ideas based on these criteria: (i) a path to scale at <$100/ton, (ii) limited competition for arable land, and (iii) a reasonably clear path to quantifying MRV and storage uncertainty.

We developed a preliminary concept for sporopollenin production using microalgae in desert-based raceway ponds. Several microalgae species naturally produce sporopollenin in their cell walls, including auxenochlorella protothecoides, haematococcus pluvialis, and dunaliella salina (in its cyst stage). Our goals were to maximize the growth rate of the algae, and the percentage of sporopollenin in the cell wall, and also to decrease the costs of the production system as much as possible by 2040.

Our progress and findings
After conducting more detailed techno-economic analyses of these systems, we found that one of the following would have to be true if sporopollenin comprised a maximum of 20% dry biomass:
- We could produce biomass so cheaply that sporopollenin could be sold for <$100/ton, implying the production of algae biomass at <$20/ton. Even in the cheapest, most productive scenario we could imagine, it does not seem possible to achieve this in a controlled environment. Biomass can likely only be grown that cheaply in an open, self-regulating environment, where ambient conditions are adequate to sustain growth (but culture contamination and competition are a constant risk). Engineered microbes in an open system would also face regulatory and MRV challenges.
- We could offset the production costs with a high-value co-product. The biggest issue here was the need for an established co-market with millions of tons of demand. Bio-fuels, bio-plastics, and the idea of massive algae food protein markets were too early and uncertain, while high-value products like astaxanthin and other algae-based supplements currently have markets of <100 tons/year.
We assumed there would be biological limitations on producing more than 20% sporopollenin in a cell. If sporopollenin was our only product, this meant we would always be stuck producing at least 80% more biomass than we intended to sell as CDR. Meanwhile, if we wanted to separate and sell the rest of the biomass, we would incur much higher separation and processing costs without a lucrative secondary market for millions of tons of algae biomass. Reflecting on this project led us to refine our understanding of the criteria that determine whether a synbio solution is likely to scale CDR at a low cost.
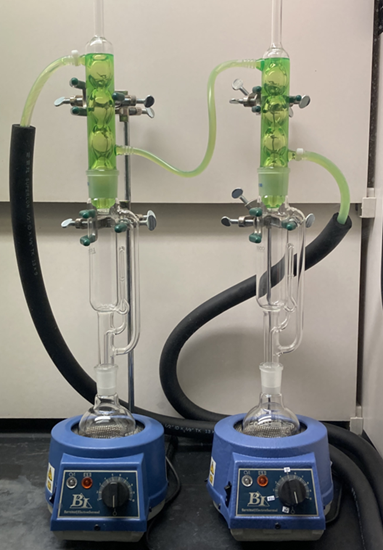
Based on our work in trees, we assumed that there would be economies of scale involved in growing megatons of biomass because live organisms are self-replicating. When we plant a forestry project, there are high up-front costs for site prep and planting, but also exponential biomass accumulation during most of the project lifetime. The algae projects we considered were different in key ways that illustrated how biological solutions are not always cheaper and more scalable. Biological solutions are most effective when they have a strong multiplier effect in terms of value created. In this case, even though the algal biomass would have grown rapidly, this did not translate to equivalent growth in sufficiently valuable output.
We developed the following graph that shows the “spectrum” of potential applications of synbio to climate grouped by input cost vs. yield:
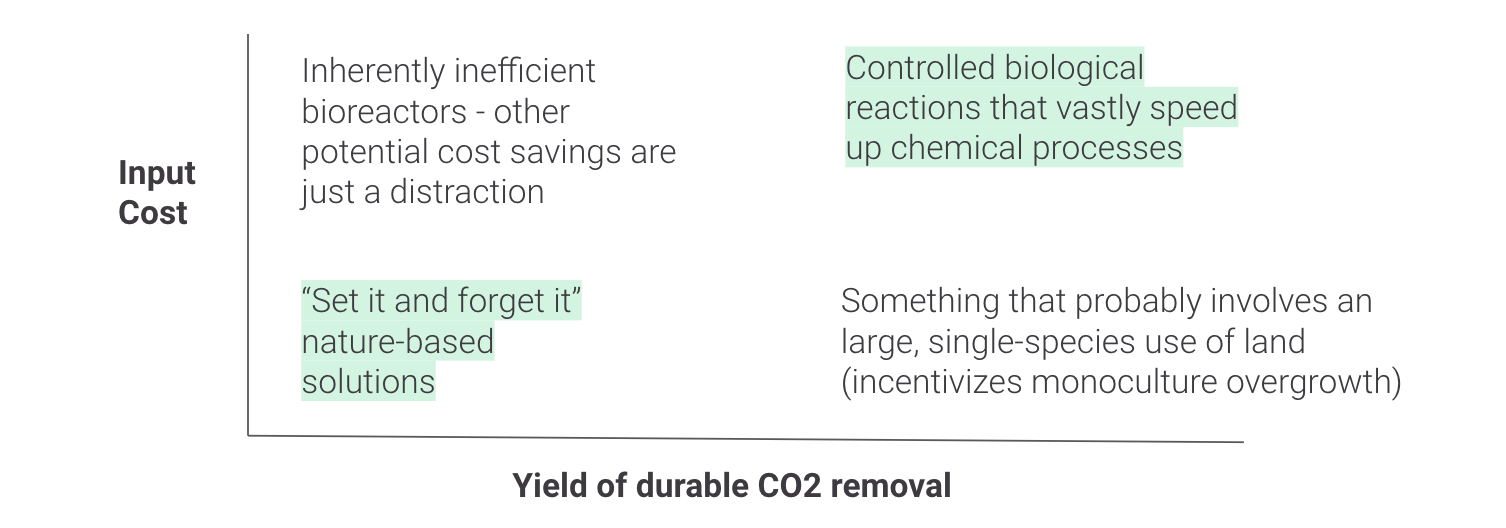
Four examples are provided to illustrate solutions along the spectrum, although these are far from the only possibilities.
“Set it and forget it” nature-based solutions can improve or restore land with low input costs over time. These are self-regulating systems that may have higher land use requirements, but the land change is positive and involves co-benefits such as biodiversity restoration. The “downside” is that prioritizing biodiversity means limiting the concentration of the target species, which can cap the reasonable per-acre yield of durable carbon storage.
Trees accumulate so much biomass overall that planting photosynthesis-enhanced trees alongside diverse other species is projected to have a large impact on additionality. However, when it comes to small plants and/or creating a substance that is only a small percentage of the plant, the risk here is creating low-cost, low-yield projects. The pitfall we recommend avoiding is trying to get around this by growing as much of the synbio species as possible per acre. At best, such a solution might require intensive maintenance. At worst, the species might become ecologically disruptive.
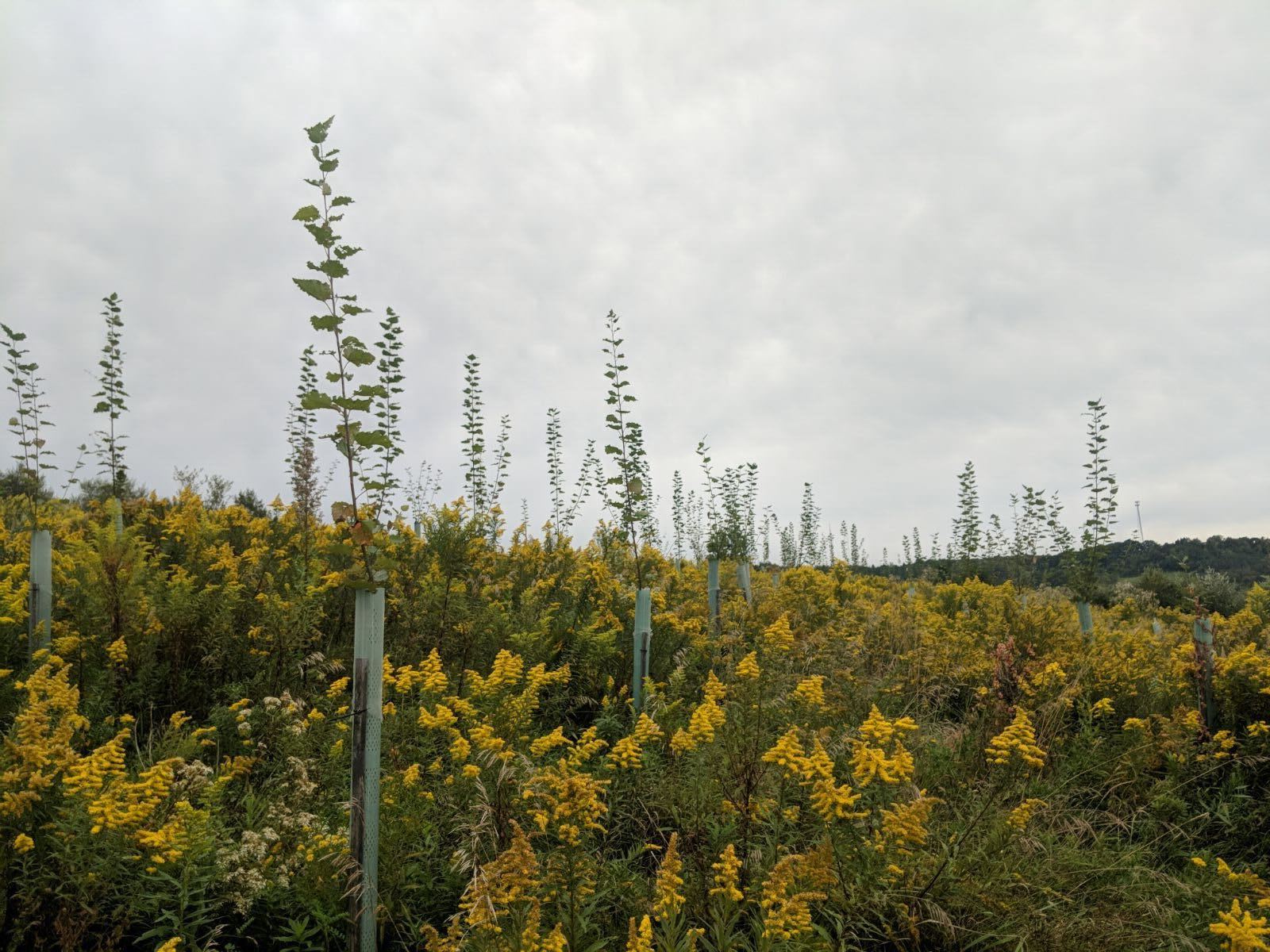
Meanwhile, certain types of engineered solutions that involve a biological component might still incur the high capital and operating costs of any other engineered solution. If high costs are incurred to maintain a controlled environment such as a bioreactor, the biological component should be held to the same output standards as a material or chemical solution. This seems straightforward, but there are situations where a high growth rate does not equal a high yield of 1000+ years of CO₂ storage. If there is a favorable ratio of primary production to CO₂ storage, we regard this as a sign that high input costs may be justified. The pitfall we recommend avoiding is relying too heavily on cost reductions in closing the cost gap, without high confidence that the synbio species is going to act as a force multiplier in terms of the CDR yield. Our observation has also been that for more complex systems, higher capital costs are also correlated with high emissions due to processing. This makes it even more important that a force multiplier relationship is introduced by the synbio trait - more linear improvements can easily be offset by added emissions associated with handling and processing the biomass.
The path forward: How can we achieve durable synbio carbon dioxide removal?
We know that nature-based solutions can scale quickly and cheaply, but only when they involve genuine restoration of self-sustaining ecosystems. If low input costs are the goal, we would start with an inventory of the most powerful carbon sinks in nature, and ask which biological interventions can increase their overall rate of carbon drawdown or slow their decomposition over time. Under this framework, we would prioritize meaningful improvements in the health and performance of forests, grasslands, peatlands, and other large-scale carbon sinks, even if there are open questions regarding the durability of these solutions. They can still act as a powerful net carbon sink as they scale to millions of acres, not to mention the ecosystem co-benefits.
We believe the challenge will be to identify opportunities where synbio can rapidly achieve permanent storage while overcoming similar versions of the same issues:
- Excess production of unrelated biomass
- Requiring a monoculture to achieve sufficient scale
- Needing highly controlled, expensive growth environments to perform adequately
- Slow and/or diffuse yield of CDR, resulting in negligible carbon yield per acre and low project returns
As we look at other applications of synbio for CDR (bio-enhanced weathering reactions, bio-enhanced ocean alkalinity enhancement, and bio-enhanced DAC), we can see potential parallel challenges. What species will be used? Will the new species singlehandedly accelerate CO₂ sequestration and storage enough to be worth it? What conditions will be employed to grow and maintain adequate population levels of the target species? Are these conditions too expensive and sensitive to justify the cost?
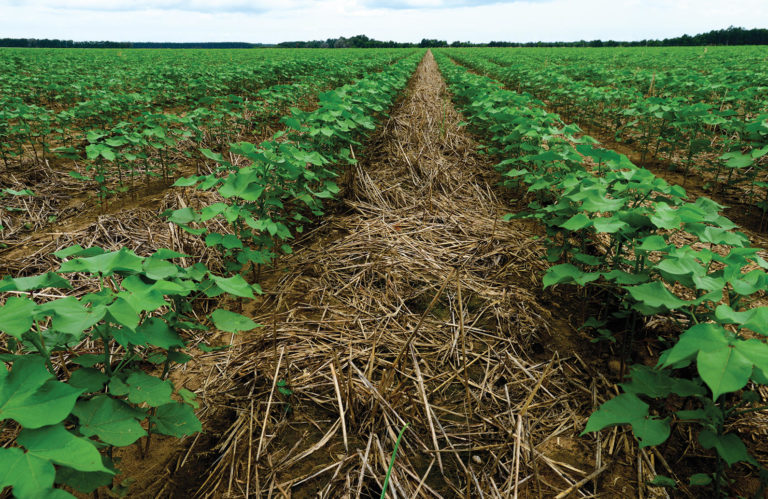
The level of excitement we see in the CDR community around synbio is motivating, and we learned through this project that there is a lot of industry demand for synbio traits to improve various processes. Because this area is still in its early stages, we invite open discussion and objective evaluation of these challenges to guide the field toward the ideas that are most likely to scale. While we’re sorry for now to say goodbye to the diamond of the plant world, our takeaway is not to become discouraged - it’s to refine our understanding of the true opportunities and limitations of biological solutions and evolve in this direction.